Tuberculosis (TB) is a major global health problem, and Mycobacterium tuberculosis (M. tuberculosis) has infected one-third of the world’s population.1,2 There were 10.4 million new cases and 1.8 million deaths due to TB in 2016.2 M. tuberculosis enters the human respiratory system through the inhalation of aerosols, typically through coughing. M. tuberculosis then colonizes inside alveolar macrophages and forms granuloma. After inhalation, M. tuberculosis is ingested by phagocytosis by resident alveolar macrophages and tissue dendritic cells.3,4 Finally, the immune cells are contributed, and the pathological mark of TB, the granuloma, is formed. In the granuloma, macrophages differentiate into epithelioid cells or foamy macrophages, or fuse to form giant cells and become surrounded by lymphocytes, fibroblasts, and extracellular matrix proteins. M. tuberculosis survives until the granuloma fails due to immunosuppression.5,6 In fact, M. tuberculosis uses the granuloma for their benefit upon initial infection, recruiting new macrophages to allow spread between host cells.7 In active tuberculosis, the caseous granuloma center contains necrotic macrophages. When the granuloma rupture, spillage of M. tuberculosis into the airways achieved, and M. tuberculosis also allows spread to new individuals.5,6
The main component of TB pathogenesis is that the bacilli survive, grow, and replicate within the host macrophages. These attributes are partly thanks to the ability of M. tuberculosis to prevent maturation of the phagosome by blocking phagosome integration with lysosomes to form the phagolysosome.8 It has been proven that the successful parasitization of macrophages is a clever action by which M. tuberculosis keeps away the immune response of the host cells.9,10
M. tuberculosis can also attenuate human immunological function through replicating within macrophages as target cells. The intracellular replication of M. tuberculosis into the macrophages finally led to the death of macrophages and the release of extracellular pathogens. Support of M. tuberculosis into the macrophages requires that the pathogen continuously set up the infection into the sensitive macrophages.10
The treatment of patients with multidrug-resistant M. tuberculosis and extensively drug resistant M. tuberculosis strains has become a serious challenge.11 The treatment of patients with TB requires long-term use of multi-drug regimens. Some of these multi-drug regimens interact with other antibacterial drugs, increasing the possibility of drug toxicity.12 Studies indicate that rifampicin can induce liver injury in mice,13 and some antitubercular drugs have hepatotoxicity effects.14 Therefore, there is always a need for simple, long-term, and effective antitubercular drug regimens to treat M. tuberculosis.
Researchers found that the mixed colloidal silver (Ag) and zinc oxide (ZnO) metallic nanoparticles (MeNPs) can inhibit M. tuberculosis H37Rv, even within THP-1 cell lines.15 In addition, mixed AgZnO nanocomposites can also eliminate H37Rv M. tuberculosis and MDR-TB after phagocytosis by the THP-1 cell lines.16 Recent studies show that mixed AgZnO nanocomposites and rifampicin have synergism effects against M. tuberculosis.16 In the last years, researchers concentrated on preparing co-delivery mixed MeNPs containing antitubercular antibiotics encapsulated to non-toxic and biodegradable polymers.17,18 Of course, the toxic effects of MeNPs on THP-1 and normal human lung cells (MCF-7 cell lines) should be considered.
Studies show that MeNPs in human tissues and cell-cultures produce several toxins that can increase the oxidative stress and production of inflammatory cytokines. MeNPs might contribute to the apoptosis of cells. MeNPs can penetrate through the cell membrane bilayer, mitochondria, and the nucleus. Thus, they might destroy the mitochondria and cause mutations in DNA. The size, dimensions, chemical composition, shape, surface structure, surface charge, density, and solubility of MeNPs are major factors determining toxicity.19 Studies also indicated that the initial concentration of MeNPs plays a key role in their toxicity against human cells, especially in THP-1 cell lines.16
This work’s major objective was to introduce MeNPs as a growth inhibitor of M. tuberculosis and inducers to phagosome maturation into the infected macrophages. Given this goal, we investigated the survival mechanisms of M. tuberculosis into macrophages and presented the novel chemicals, phytogenic, and encapsulated mono-metallic or bimetallic Ag, ZnO, and gold (Au) antitubercular MeNPs to inhibition of intracellular M. tuberculosis and gallium (Ga) MeNPs to enhance phagosome maturation. We also evaluated the role of magnetic iron (Fe) MeNPs as nanocarriers of antituberculosis drugs.
M. tuberculosis pathogenesis
TB is known as an airborne disease caused by M. tuberculosis. M. tuberculosis and M. tuberculosis complex species, including M. bovis, M. africanum, M. microti, M. caprae, M. pinnipedii, M. canetti, and M. mungi may cause disease in humans.20
Mostly, TB infection arises when people suffering from pulmonary or laryngeal TB disease have a cough, sneeze, shout, or sing, and another person inhales droplets, including TB bacilli. TB bacilli then try to reach the alveoli of the lungs through the upper respiratory tract and bronchi. The infected droplets are around 1–5 mm.21 After that, bacilli are phagocytosed to form tubercles in the tissue-resident alveolar macrophages to the inflamed region. Under the week host defense conditions, bacilli may survive for a long time as a source of post-primary infection into the alveolar macrophage.22 In fact, macrophages that failed to kill the mycobacterial invaders produced chemo-attractants such as chemokines.23 Chemokines were produced by resident alveolar macrophage and pneumocytes, which invite neutrophils, monocyte-derived macrophages, NK cells, and T cells, which increase inflammation.23 Then, the cells formed hard shell structures known as granulomas. In this condition, the tubercle bacilli remain in the ‘dormant state’ inside macrophages in the granulomas for years [Figure 1].
In the active form of TB, bacilli can also replicate into the host macrophage, break down the macrophage cell membrane, and is released outside of it.22 The bacilli released from the demolished macrophage move around through the lymphatic system and bloodstream to the brain, larynx, lymph nodes, lung, spine, bones, or kidneys, which is known as military TB.21
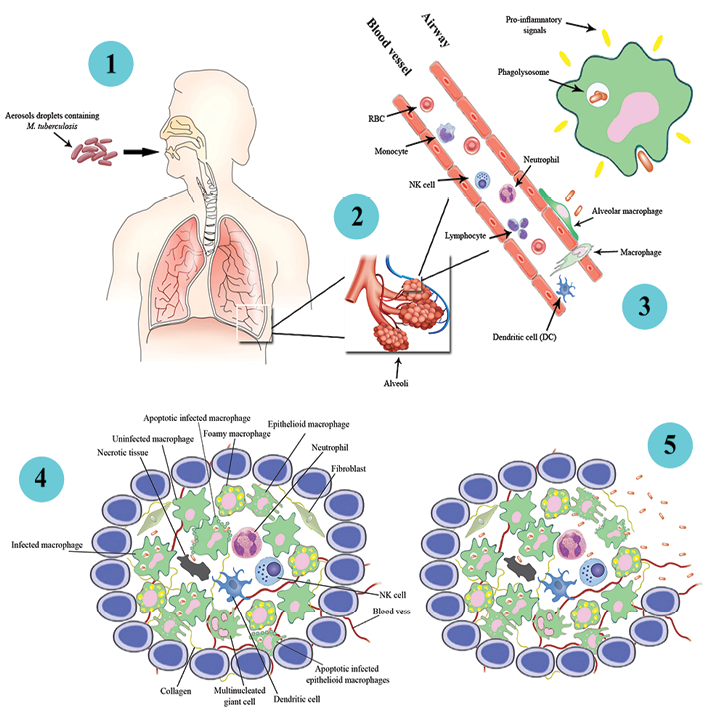
Figure 1: The infection pathways of Mycobacterium tuberculosis in human macrophage cells leading to the formation of a granuloma (1-4). Resident alveolar macrophages phagocytes inhaled M. tuberculosis and products the pro-inflammatory response and recruitment of fibroblasts, lymphocytes, neutrophils, natural killer (NK) cells, collagens, necrotic tissues, dendritic cells, foamy macrophages, infected apoptotic macrophage, infected apoptotic epithelial macrophages, and epithelial macrophages, and the formation of a granuloma. However, if the human immune system for any reason is weakened, M. tuberculosis is activated and replicated within the granuloma structure. In this case, the necrotic nucleus of granuloma develops. When the number of M. tuberculosis is increased within the granuloma, it ruptures, and M. tuberculosis is spilled into the airways. Each of the bacteria has the potential to infect other alveolar macrophages to the formation of a new granuloma.
Antibiotics therapy challenges in the treatment of M. tuberculosis into the macrophage
There are several issues in terms of the efficiency of anti-TB antibiotics. First, there is no direct correlation between the plasma concentration of anti-TB antibiotics and its intracellular concentration. Furthermore, the intracellular activity of anti-TB antibiotics is justified by the rate of internalization, excretion rate, cell transformation, and pH.24 Moreover, anti-TB antibiotics have a flexible internalization and intracellular accumulation path.24 In addition, anti-TB drugs may not penetrate granulomas.7 One of the other critical challenges is the limitation of the administration routes and the prolongation of TB treatment.15 We know the intracellular survival of M. tuberculosis in the host cell pertains to its adaptation-evading of the immune system, dissemination, and selection of aggressive and multi-drug resistant (MDR-TB).24 Studies have shown that the azithromycin could accumulate completely in phagolysosomes; however, it had vastly low antimicrobial efficiency on pathogens. Comparably, moxifloxacin was not only unable to accumulate in the phagolysosome but also has high antimicrobial efficiency.25 Cellular accumulation of antibiotics and some local environmental conditions (such as pH) are important parameters for intracellular activity.25
Survive mechanisms of M. tuberculosis in macrophages
Knowing the intracellular adaptive mechanisms for the survival of M. tuberculosis into the macrophages is essential in formulating convenient therapies. M. tuberculosis has adaptive mechanisms that run away from the human immune system, especially macrophages. These mechanisms will be discussed below.
prevention the fusion of primary phagosomes with lysosomes
M. tuberculosis can interfere with the transformation of the primary endosomes and phagosome maturation, and subsequently, the fusion with lysosomes is delayed or blocked [Figure 2].26
M. tuberculosis also can prevent the transformation of primary endosomes into phagolysosome by the reduction of levels of proton ATPase inside the endosomes. M. tuberculosis can prohibit connecting of the inducible nitric oxide synthase (iNOS).27,28 Studies show that M. tuberculosis is also able to eliminate the phosphatidylinositol 3-phosphate29 [Figure 2].
demolition of endosomes or early phagosomes walls and escape into the cytosol
M. tuberculosis can elude from the degradation in phagolysosomes by demolishing the endocytic vesicle wall and entering the cytosol. Escaping the endocytic vesicle is an essential step in the intra-macrophage survival for M. tuberculosis in the cytosol [Figure 2].30 In 2003, Jafari et al,19 recorded images showing some of the H37Rv strain of M. tuberculosis escaping from the phagolysosomes and arriving in the cytosol [Figure 2].
Brown et al,31,32 discovered that M. marinum is also able to escape from its phagolysosome and move around by the motive force of actin through Arp2/3 complex-mediated actin reorganization dependent on activation of WASP. Besides this, proteins secreted by M. tuberculosis, such as ESAT-6, play important roles in virulence. Escaping from the phagosome depends on ESAT-6 alone or in complex with its chaperone CFP-10 (ESAT-6: CFP-10) [Figure 2].33
These proteins lead to increased replication rates in the cell cytoplasm.34 In addition, more likely to the mycobacterial cell envelope can be mistaken for a phagosome membrane due to its lipid-rich structures.35 One explanation for the presence of cytosolic M. tuberculosis in some preparations is that it exists of host triacylglycerol in both the prevention of phagosome maturation and persistence in granulomatous lesions.6
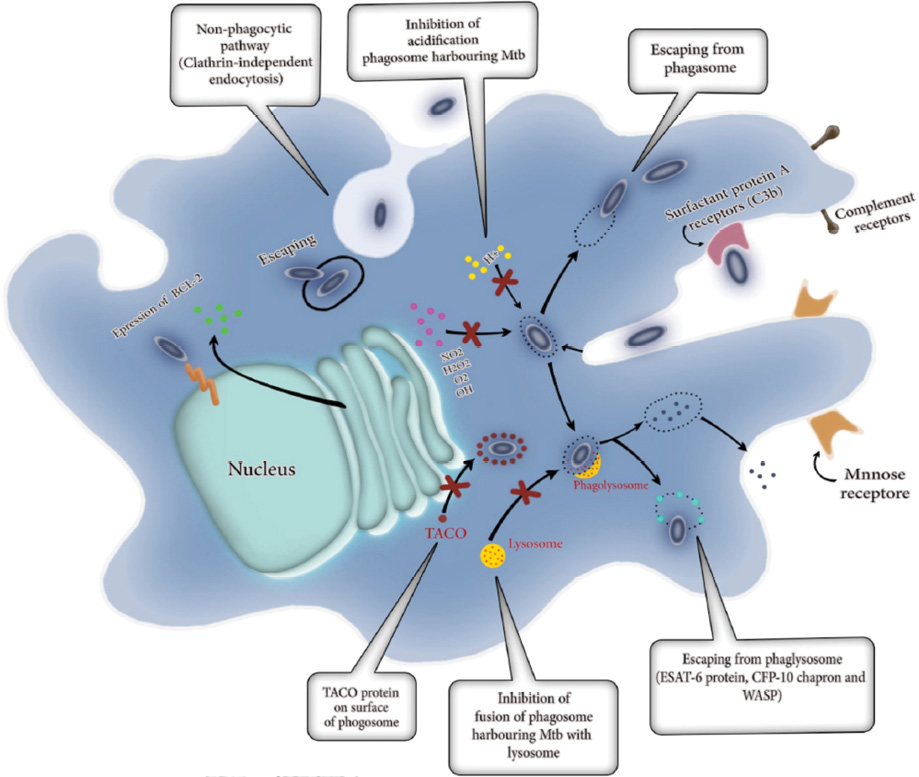
Figure 2: Survival and adaptive mechanisms of Myobacterium tuberculosis in macrophages. Some M. tuberculosis can enter into the alveolar macrophages through a non-phagocytic pathway called clathrin-independent endocytosis. M. tuberculosis can then escape from phagosomes and release into the cytosol of macrophages. M. tuberculosis can induce the expression of anti-apoptosis genes (Bcl-2) into the macrophages. The absorption of H+, H2O2, O2, NO2, and OH also are inhibited to control the acidification of phagosome harboring M. tuberculosis. M. tuberculosis can escape from phagolysosome by producing ESAT-6 proteins, Wiskott-Aldrich syndrome protein (WASP), and CFP-10 chaperone. M. tuberculosis prevents transforming of primary endosomes in phagolysosome via the reducing of levels of proton ATPase inside the endosomes, connecting the inducible iNOS and elimination of the phosphatidylinositol 3-phosphate (PI3P). Tryptophan-aspartate containing coat (TACO) proteins, represents a component of the phagosome coat that is released earlier than phagosome fusion with or maturation into lysosomes. In macrophages containing TACO, it leads to preventing to forming phagolysosome and following that M. tuberculosis can survive within the phagosome.
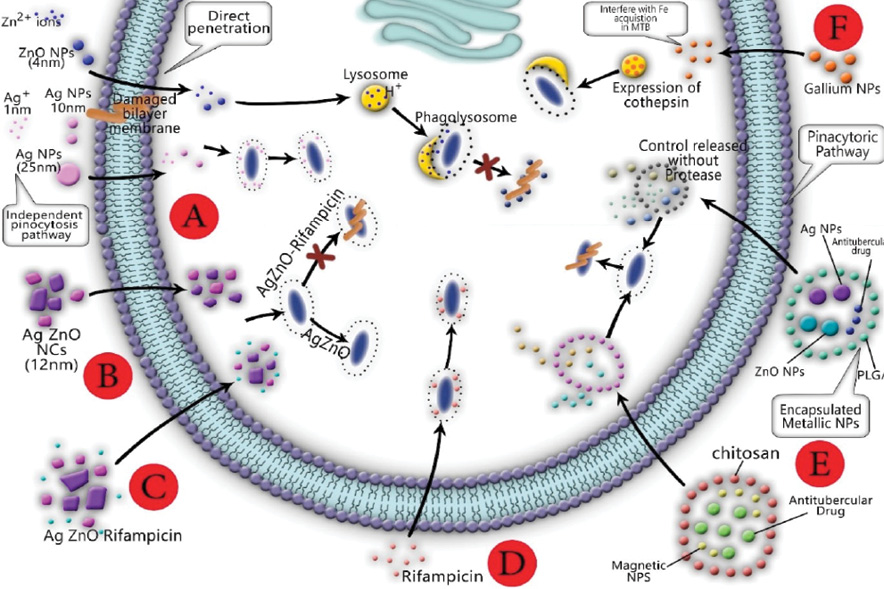
Figure 3: Colloidal ZnO nanoparticles (NPs) can penetrate the bilayer membrane directly and accumulate in lysosomes. (A) Lysosomes containing ZnO NPs are integrated into infected phagosomes and eliminate M tuberculosis; colloidal Ag NPs alone cannot kill M. tuberculosis. (B) Opposed to mixture Ag/ZnO nanocrystals. (C, D) Ag/ZnO-rifampicin is able to eliminate M. tuberculosis into the phagosome. (E) Encapsulated magnetic NPs and antibiotics loaded polymers import to macrophage by endocytosis and subsequently release NPs and antibiotics in the cytosol. Mixed magnetic NPs and antitubercular NPs are able to kill M. tuberculosis into the macrophage. (F) The macrophage infected with M. tuberculosis presented an expression of cathepsin D, which plays an important role in macrophage activation.
internalization in macrophages by non-phagocytic pathways which are not likely coupling with lysosomes
Internalization of M. tuberculosis in macrophages by non-phagocytic pathways involves interactions between M. tuberculosis and the membrane of the macrophages causing the formation of vesicles.36 Macrophage’s internalization is achieved by coupling of M. tuberculosis with lipid rafts and receptors of which mediate a non-phagocytic endocytosis.36 Studies indicated that the synthetic antimicrobial polymers have the ability to induce membrane lysis and bind to the genomic material of mycobacteria, thereby inducing mycobacterial cell death and was also able to kill the intracellular mycobacteria effectively without inducing any toxicity to mammalian cells.37 Yavvari et al,37 showed that synthetic antimicrobial polymers has clathrin-independent penetration and escape from hydrolytic lysosomal degradation and effectively kill the intracellular bacteria. M. tuberculosis also can interact with the macrophage apoptotic mechanisms to increase expression of anti-apoptotic molecules such as Bcl2 [Figure 2].38
The entrance pathway of particles into the macrophage
Macrophages have the ability to engulf opsonized NPs, modified mannose, IgG, and many complements larger than 500 nm, including M. tuberculosis39 from phagocytic pathways.40 Macro-pinocytosis is an actin-dependent pinocytic pathway by which macrophages can engulf droplets of extracellular fluid within large vacuoles formed by the fusion of a plasma membrane extension with non-extended plasma membrane [Figure 3]. It may be more likely that the macro-pinocytosis pathway is for the swallowing of agglomerated particles. In other words, the macrophage can use macro-pinocytosis pathways to engulf agglomerated particles, ligand-modified NPs, viral NPs, and polyethylene glycol NPs.41 Studies indicated that ligand-modified NPs and some viral NPs can enter into the macrophage by specific receptors such as clathrin-mediated, caveolin-mediated, or clathrin/caveolin-mediated pinocytosis.41
Interestingly, MeNPs and dendrimers with dimensions between 4 and 10 nm can directly penetrate macrophages [Figure 3]. Based on the transmission electron microscopy images of previous study, THP-1 cells exposed to Ag and ZnO NPs could penetrate the cell bilayer membrane and enter the cell [Figure 3].
The role of phagosomes maturation in antitubercular properties
The phagocytosis of M. tuberculosis is thanks to the role of receptor-mediated phagocytosis of macrophages. After phagocytosis of M. tuberculosis, the phagosome is formed, and subsequently, a series of fusion events is achieved into endocytic pathways called 'phagosomal maturation'. The phagosome of which interact with endosomes and then lysosomes obtain a wide range of antimicrobial properties designed to completely destroy M. tuberculosis. The phagosomal maturation stages in macrophages can be identified based on the proteins present on the phagosomal membrane. In fact, the proteins correspond to the endosome type with which the phagosome has interacted.24 The connection between the phagosome and endosomal network and lysosomes have two hypotheses; the kiss and run-hypothesis and the fusion hypothesis.24 In addition, phagosomes can obtain hydrolases from the 6-thioguanine nucleotide. A microtubule-based transport system also is more likely to be responsible for providing the scaffold for endosome movement. In addition, membrane rafts and glycol lipoprotein microdomains play a key role in phagosomal maturation. The fusion and fission events eventually occur in an oxidative, acidic, and degradative phagolysosome environment, which is designed to eliminate invading microbes effectively.24 In fact, the main antimicrobial mechanisms of the mature macrophage phagosome are as a result of acidification, activation of the NADPH oxidase NOX2, activation of iNOS, antimicrobial peptides, and protein degradation [Figure 3].42
The intercellular antitubercular impact of mono-metallic/bimetallic nanoparticles and nano metallic carriers
The MeNPs are accepted as antitubercular agents that destroy the integrity of bacterial membranes.15 The MeNPs are potentially capable of attaching and sticking to the cell wall of M. tuberculosis and subsequently destroy it, mechanically.15 In general, the toxicity of MeNPs is thanks to oxidative stress and free radicals called reactive oxygen species.43 Conceptually, antitubercular mechanisms of MeNPs arrived from the interaction between the M. tuberculosis and NPs, as well the bio-reactive properties of the dissolved ionic fraction [Figure 3].44–47
Ag and ZnO NPs are considered antibacterial agents against pathogenic bacteria.48 It was reported that Ag NPs increase bacterial cell permeability by motiving the aggregation of proteins in the periplasmic space, forming nano-sized pores in the bacterial membrane. Increasing bacterial membrane permeability may facilitate increased penetration of intracellular antibiotics.49 Zn is a natural metal used by alveolar macrophages to increase bactericidal pressure against internalized M. tuberculosis within the endosome. For this reason, ZnO NPs can interact with the membrane of bacteria, which leads to the formation of surface pores and the release of intracellular nucleotides.49 To date, many researchers have synthesized the colloidal,19 nanocrystals,16 and encapsulated49,50 antitubercular Ag and ZnO NPs by chemical and biological methods [Figure 3].51
chemical mono/bimetallic silver and zinc oxide antitubercular nanoparticles
In 2017, Jafari et al,52 synthesized colloidal Ag and ZnO NPs using chemical reduction and deposition methods. The average size of colloidal Ag and ZnO NPs was estimated at 13±3.14 nm and 4±0.88 nm. They reported that the colloidal Ag NPs were not able to eliminate intracellular M. tuberculosis into the macrophages, completely (minimum inhibitory concentration (MIC) ≥ 25 ppm).19 Whereas, the colloidal ZnO NPs demonstrated antitubercular behavior in the macrophage against M. tuberculosis H37Rv,19 but it was toxic against MCF-7 cell lines ex-vivo. Based on this assumption, 0.663 ppm of 5Ag:5ZnO not only was able to kill M. tuberculosis H37Rv but also showed the lowest cytotoxicity effects on MCF-7 and THP-1 cell lines.19 They also synthesized a mixture of Ag/ZnO nanocrystals by oxalic reduction method with an average size of 12 nm. The results showed that mixture nanocrystals were not able to eliminate H37Rv strain of M. tuberculosis ex-vivo.44 It seems more likely that the ability of metallic colloidal NPs to penetrate macrophages is more than agglomerated metallic nanocrystals. Furthermore, the antituberculosis properties of MeNPs likely arise from the formation of metal ions in the aqueous medium, and subsequently due to penetration into the macrophage [Figure 3].53
encapsulated of silver and zinc antitubercular nanoparticles
Studies show that Ag and ZnO NPs encapsulated into a biodegradable and biocompatible polymer such as poly lactic-co-glycolic acid (PLGA), forms a multi-metallic microparticle. Encapsulated NPs have the ability to transport antibiotics into M. tuberculosis-infected macrophages. Co-delivery of Ag and ZnO NPs into a larger micron-sized carrier collaborated to selective metallic/metal oxide NPs uptake by macrophages through passive targeting, initial burst release of ions from the encapsulated Ag and ZnO NPs, and eventually, reduction of metallic/metal oxide NPs toxicity [Figure 3].49,50 Pati et al,17 in 2016, synthesized Zn and rifampicin, and encapsulated it into transferrin-conjugated Ag quantum-dots to improve delivery in macrophages. They found that encapsulated Zn and rifampicin into the transferrin-conjugated Ag quantum-dots NPs play a pivotal role in increasing antitubercular activity compared to Zn-rifampicin, rifampicin, and Zn [Figure 3].17
phytogenic mono-metallic/bimetallic silver, zinc, and gold antitubercular nanoparticles
Biosynthesis of antitubercular MeNPs should be taken into consideration due to its economic feasibility, low toxicity, and simplicity of the procedure.51 Punjabi et al,51 synthesized extracellular Ag and ZnO NPs with an average size of 40 and 60 nm by Pseudomonas hibiscicola. The MICs value in both of Ag and Zn NPs against H37Rv strain and MDR strain of M. tuberculosis were reported as 1.25 mg/mL.51 Banu and Rathod reported antituberculosis activity of biosynthesized Ag NPs against M. tuberculosis and clinical isolates of MDR-TB.54 Ag NPs were biosynthesized utilizing the alcoholic extract of Plumbago auriculata and used to investigate the antitubercular effects against M. tuberculosis.55 Ag NPs with an average size of 15-45 nm showed antitubercular activity with a MIC value of 1.6 μg/mL.55 In 2016, Singh et al,56 synthesized Ag, Au, and bimetallic Ag/Au NPs using Barleria prionitis leaf extract, Plumbago zeylanica root extract, and Syzygium cumini bark extract.
The Ag NPs synthesized using the Psidium guajava leaf extract demonstrated an inhibitory effect on M. tuberculosis.56 Bimetallic Ag/Au NPs were able to inhibit 90% of mycobacterial growth at 3 μg/mL, and it presented great mycobactericidal potency in contrast with Ag NPs alone.56 Bimetallic Ag/Au NPs possessed the high efficiency to inhibit M. tuberculosis ex-vivo THP-1 infection model [Figure 3].56,57
gallium nanoparticles
The antimicrobial activity of gallium nanoparticles has been investigated against M. tuberculosis10,58 and nontuberculous mycobacterial pathogens, M. avium complex, and M. abscessus.59 Gallium and Fe have similar chemical properties. Gallium can interfere with Fe acquisition by microorganisms.10 Recently, the blocking of Fe acquisition by the gallium compound in M. tuberculosis is confirmed. Gallium has the ability to reduce the growth of intracellular M. tuberculosis into the macrophage.10 A report stated that gallium NPs can also interfere with Fe acquisition in M. tuberculosis and inhibit intracellular growth within macrophages.10 Another study reported that gallium NPs show significant growth inhibition of M. tuberculosis in THP-1 macrophages on days three and six after infection.10 Macrophages infected with M. tuberculosis H37Ra exhibited significantly higher expression of cathepsin D – an aspartic endoprotease that is distributed in lysosomes – compared to galactin 3, a member of the beta-galactoside-binding protein family that plays an important role in macrophage activation as well.10 The authors of the study found that uptake of gallium nanoparticles by macrophage led to the promotion of phagosome maturation and subsequently led to increase the antituberculosis effects of the macrophage.10 To sum up, gallium nanoparticles can deliver a drug to macrophages, inhibit M tuberculosis and nontuberculous mycobacterial growth,59 and reduce the inhibition of phagosome maturation [Figure 3].10
iron nanoparticles as a nanocarrier
Iron, an essential nutrient for nearly all living cells, plays a critical role in many important enzymatic reactions as a cofactor. Its ability to redox cycle between Fe(II)/Fe(III) enhances electron transfer.10 In humans, Fe is tightly bound to transferrin, lactoferrin, ferritin, and heme. Pathogenic bacteria must acquire Fe mainly from these Fe complexing proteins for growth and metabolism. Many pathogens possess highly efficient Fe uptake mechanisms. These bacteria release Fe solubilizing (chelating) compounds, siderophores, to obtain Fe (III) from host Fe-binding molecules for growth. Carboxymycobactin and mycobactin are mycobacterial siderophores that chelate Fe (III) extracellularly and intracellularly, and are critical to the virulence of these organisms in vitro and in vivo.10
M. tuberculosis within the phagosomes of macrophages can acquire Fe from extracellular transferrin and sources within the macrophage.60 Lu et al,61 in 2018, synthesized encapsulated Fe3O4/hyperbranched polyester-(2-dodecen-1-yl) succinic anhydride2-Dodecen-1-/isoniazid magnetic NPs with controlled drug release characteristics. They suggested that this encapsulated microparticle containing Fe exhibited good drug release properties and might be suitable to treat TB. In 2017, Saifullah et al,62 designed a novel antitubercular multifunctional formulation containing fabricating graphene oxide with iron oxide magnetite NPs as a nanocarrier of ethambutol. They found this formulation had prolonged sustained release of ethambutol and could reduce the dosing frequency for the treatment of M. smegmatis compared to ethambutol alone.62 El Zowalaty et al,63 prepared streptomycin-loaded, chitosan-coated magnetic NPs. Remarkably, the MIC of this formulation against M. tuberculosis was 732 µg/mL [Figure 3].
Conclusion
A real therapeutic advance in terms of elimination of intracellular M. tuberculosis can be done merely by fulfilling careful studies in the field of nano-therapy. Using metal NPs in therapies of respiratory infection may contribute to achieving effective TB treatment. The key role of MeNPs in medicine are diagnosis and target therapy; however, it seems that the time has come to use of antitubercular MeNPs in the treatment of intercellular M. tuberculosis. MeNPs and metal ions can penetrate throughout bilayer infected macrophage membrane due to the small size and relative mobility. They can also freely attach to M. tuberculosis of which immerse in the cytosol and even may penetrate phagolysosomes. Mixing of colloidal MeNPs can also be a good way to increase antibacterial properties and, in turn, reduce the toxicity of MeNPs to human cells. In addition, a mixture of MeNPs can be considered suitable for co-delivery along with antituberculosis drugs to eliminate intracellular M. tuberculosis into the macrophages and even granuloma. Further advances are needed in terms of changing the concept of nanotechnology into a realistic medical application as the next generation of intracellular antituberculosis drugs.
Disclosure
The authors declared no conflicts of interest. No funding was received for this study.
references
- World Health Organization. Global tuberculosis control: epidemiology, strategy, financing: WHO report 2009; 2009.
- 2. Vieira AC, Magalhães J, Rocha S, Cardoso MS, Santos SG, Borges M, et al. Targeted macrophages delivery of rifampicin-loaded lipid nanoparticles to improve tuberculosis treatment. Nanomedicine (Lond) 2017 Dec;12(24):2721-2736.
- 3. Korbel DS, Schneider BE, Schaible UE. Innate immunity in tuberculosis: myths and truth. Microbes Infect 2008 Jul;10(9):995-1004.
- 4. North RJ, Jung Y-J. Immunity to tuberculosis. Annu Rev Immunol 2004;22:599-623.
- 5. Russell DG. Who puts the tubercle in tuberculosis? Nat Rev Microbiol 2007 Jan;5(1):39-47.
- 6. de Chastellier C. The many niches and strategies used by pathogenic mycobacteria for survival within host macrophages. Immunobiology 2009;214(7):526-542.
- 7. Davis JM, Ramakrishnan L. The role of the granuloma in expansion and dissemination of early tuberculous infection. Cell 2009 Jan;136(1):37-49.
- 8. Chua J, Vergne I, Master S, Deretic V. A tale of two lipids: Mycobacterium tuberculosis phagosome maturation arrest. Curr Opin Microbiol 2004 Feb;7(1):71-77.
- 9. Choi SR, Larson MA, Hinrichs SH, Bartling AM, Frandsen J, Narayanasamy P. Discovery of bicyclic inhibitors against menaquinone biosynthesis. Future Med Chem 2016 Jan;8(1):11-16.
- 10. Choi SR, Britigan BE, Moran DM, Narayanasamy P. Gallium nanoparticles facilitate phagosome maturation and inhibit growth of virulent Mycobacterium tuberculosis in macrophages. PLoS One 2017 May;12(5):e0177987.
- 11. Oni T. Patterns of HIV, TB, and non-communicable disease multi-morbidity in an informal peri-urban setting in Cape Town, South Africa. 2015, University of Cape Town.
- 12. Golub JE, Cohn S, Saraceni V, Cavalcante SC, Pacheco AG, Moulton LH, et al. Long-term protection from isoniazid preventive therapy for tuberculosis in HIV-infected patients in a medium-burden tuberculosis setting: the TB/HIV in Rio (THRio) study. Clin Infect Dis 2015 Feb;60(4):639-645.
- 13. Kim J-H, Nam WS, Kim SJ, Kwon OK, Seung EJ, Jo JJ, et al. Mechanism investigation of rifampicin-induced liver injury using comparative toxicoproteomics in mice. Int J Mol Sci 2017 Jul;18(7):1417.
- 14. Ramappa V, Aithal GP. Hepatotoxicity related to antituberculosis drugs: mechanisms and management. J Clin Exp Hepatol 2013 Mar;3(1):37-49.
- 15. Jafari AR, Mosavi T, Mosavari N, Majid A, Movahedzade F, Tebyaniyan M, et al. Mixed metal oxide nanoparticles inhibit growth of Mycobacterium tuberculosis into THP-1 cells. Int J Mycobacteriol 2016 Dec;5(Suppl 1):S181-S183.
- 16. Jafari A, Jafari Nodooshan S, Safarkar R, Movahedzadeh F, Mosavari N, Novin Kashani A, et al. Toxicity effects of AgZnO nanoparticles and rifampicin on Mycobacterium tuberculosis into the macrophage. J Basic Microbiol 2018 Jan;58(1):41-51.
- 17. Pati R, Sahu R, Panda J, Sonawane A. Encapsulation of zinc-rifampicin complex into transferrin-conjugated silver quantum-dots improves its antimycobacterial activity and stability and facilitates drug delivery into macrophages. Sci Rep 2016 Apr;6:24184.
- 18. Kalluru, R, Fenaroli F, Westmoreland D, Ulanova L, Maleki A, Roos N, et al. Polylactide-co-glycolide-rifampicin-nanoparticles efficiently clear Mycobacterium bovis BCG infection in macrophages and remain membrane-bound in phago- lysosomes. J Cell Sci, 2013;126(14):3043-3054.
- 19. Jafari A, Mosavari N, Movahedzadeh F, Nodooshan SJ, Safarkar R, Moro R, et al. Bactericidal impact of Ag, ZnO and mixed AgZnO colloidal nanoparticles on H37Rv Mycobacterium tuberculosis phagocytized by THP-1 cell lines. Microb Pathog 2017 Sep;110:335-344.
- 20. Ashford DA, Whitney E, Raghunathan P, Cosivi O. Epidemiology of selected mycobacteria that infect humans and other animals. Rev Sci Tech 2001 Apr;20(1):325-337.
- 21. Muttil P, Wang C, Hickey AJ. Inhaled drug delivery for tuberculosis therapy. Pharm Res 2009 Nov;26(11):2401-2416.
- 22. Pham D-D, Fattal E, Tsapis N. Pulmonary drug delivery systems for tuberculosis treatment. Int J Pharm 2015 Jan;478(2):517-529.
- 23. Ehlers S, Schaible UE. The granuloma in tuberculosis: dynamics of a host-pathogen collusion. Front Immunol 2013 Jan;3:411.
- 24. Welin A. Survival strategies of Mycobacterium tuberculosis inside the human macrophage. 2011, Linköping University Electronic Press.
- 25. Seral C, Carryn S, Tulkens PM, Van Bambeke F. Influence of P-glycoprotein and MRP efflux pump inhibitors on the intracellular activity of azithromycin and ciprofloxacin in macrophages infected by Listeria monocytogenes or Staphylococcus aureus. J Antimicrob Chemother 2003 May;51(5):1167-1173.
- 26. Vergne I, Chua J, Lee HH, Lucas M, Belisle J, Deretic V. Mechanism of phagolysosome biogenesis block by viable Mycobacterium tuberculosis. Proc Natl Acad Sci U S A 2005 Mar;102(11):4033-4038.
- 27. Sturgill-Koszycki S, Schlesinger PH, Chakraborty P, Haddix PL, Collins HL, Fok AK, et al. Lack of acidification in Mycobacterium phagosomes produced by exclusion of the vesicular proton-ATPase. Science 1994 Feb;263(5147):678-681.
- 28. Miller BH, Fratti RA, Poschet JF, Timmins GS, Master SS, Burgos M, et al. Mycobacteria inhibit nitric oxide synthase recruitment to phagosomes during macrophage infection. Infect Immun 2004 May;72(5):2872-2878.
- 29. Vergne I, Fratti RA, Hill PJ, Chua J, Belisle J, Deretic V. Mycobacterium tuberculosis phagosome maturation arrest: mycobacterial phosphatidylinositol analog phosphatidylinositol mannoside stimulates early endosomal fusion. Mol Biol Cell 2004 Feb;15(2):751-760.
- 30. Jamwal SV, Mehrotra P, Singh A, Siddiqui Z, Basu A, Rao KV. Mycobacterial escape from macrophage phagosomes to the cytoplasm represents an alternate adaptation mechanism. Sci Rep 2016 Mar;6:23089.
- 32. Stamm LM, Morisaki JH, Gao LY, Jeng RL, McDonald KL, Roth R, et al. Mycobacterium marinum escapes from phagosomes and is propelled by actin-based motility. J Exp Med 2003 Nov;198(9):1361-1368.
- 33. Stamm LM, Pak MA, Morisaki JH, Snapper SB, Rottner K, Lommel S, et al. Role of the WASP family proteins for Mycobacterium marinum actin tail formation. Proc Natl Acad Sci U S A 2005 Oct;102(41):14837-14842.
- 34. Sreejit G, Ahmed A, Parveen N, Jha V, Valluri VL, Ghosh S, et al. The ESAT-6 protein of Mycobacterium tuberculosis interacts with beta-2-microglobulin (β2M) affecting antigen presentation function of macrophage. PLoS Pathog 2014 Oct;10(10):e1004446.
- 35. van der Wel N, Hava D, Houben D, Fluitsma D, van Zon M, Pierson J, et al. M. tuberculosis and M. leprae translocate from the phagolysosome to the cytosol in myeloid cells. Cell 2007 Jun;129(7):1287-1298.
- 36. Zuber B, Chami M, Houssin C, Dubochet J, Griffiths G, Daffé M. Direct visualization of the outer membrane of mycobacteria and corynebacteria in their native state. J Bacteriol 2008 Aug;190(16):5672-5680.
- 37. Sibley DL, Charron AJ, Hakansson S, Mordue DG. Invasion and intracellular survival by Toxoplasma. 2013.
- 38. Yavvari PS, Gupta S, Arora D, Nandicoori VK, Srivastava A, Bajaj A. Clathrin-independent killing of intracellular mycobacteria and biofilm disruptions using synthetic antimicrobial polymers. Biomacromolecules 2017 Jul;18(7):2024-2033.
- 39. Ríos-Barrera VA, Campos-Peña V, Aguilar-León D, Lascurain LR, Meraz-Ríos MA, Moreno J, et al. Macrophage and T lymphocyte apoptosis during experimental pulmonary tuberculosis: their relationship to mycobacterial virulence. Eur J Immunol 2006 Feb;36(2):345-353.
- 40. Aderem A, Underhill DM. Mechanisms of phagocytosis in macrophages. Annu Rev Immunol 1999;17(1):593-623.
- 41. Hamilton RF, Buckingham S, Holian A. The effect of size on Ag nanosphere toxicity in macrophage cell models and lung epithelial cell lines is dependent on particle dissolution. Int J Mol Sci 2014 Apr;15(4):6815-6830.
- 42. Kruth HS. Receptor-independent fluid-phase pinocytosis mechanisms for induction of foam cell formation with native LDL particles. Curr Opin Lipidol 2011;22(5):386.
- 43. Flannagan RS, Cosío G, Grinstein S. Antimicrobial mechanisms of phagocytes and bacterial evasion strategies. Nat Rev Microbiol 2009 May;7(5):355-366.
- 45. Nel AE, Mädler L, Velegol D, Xia T, Hoek EM, Somasundaran P, et al. Understanding biophysicochemical interactions at the nano-bio interface. Nat Mater 2009 Jul;8(7):543-557.
- 46. Ivask A, Elbadawy A, Kaweeteerawat C, Boren D, Fischer H, Ji Z, et al. Toxicity mechanisms in Escherichia coli vary for silver nanoparticles and differ from ionic silver. ACS Nano 2014 Jan;8(1):374-386.
- 47. Bondarenko O, Ivask A, Käkinen A, Kurvet I, Kahru A. Particle-cell contact enhances antibacterial activity of silver nanoparticles. PLoS One 2013 May;8(5):e64060.
- 48. Morones JR, Elechiguerra JL, Camacho A, Holt K, Kouri JB, Ramírez JT, et al. The bactericidal effect of silver nanoparticles. Nanotechnology 2005 Oct;16(10):2346-2353.
- 49. Martínez-Gutierrez F, Thi EP, Silverman JM, de Oliveira CC, Svensson SL, Vanden Hoek A, et al. Antibacterial activity, inflammatory response, coagulation and cytotoxicity effects of silver nanoparticles. Nanomedicine 2012 Apr;8(3):328-336.
- 50. Aderibigbe BA. Metal-based nanoparticles for the treatment of infectious diseases. Molecules 2017 Aug;22(8):1370.
- 51. Ellis T, Chiappi M, García-Trenco A, Al-Ejji M, Sarkar S, Georgiou TK, et al. Multimetallic microparticles increase the potency of rifampicin against intracellular Mycobacterium tuberculosis. ACS Nano 2018 Jun;12(6):5228-5240.
- 52. Xiang Y, Li J, Liu X, Cui Z, Yang X, Yeung KW, et al. Construction of poly(lactic-co-glycolic acid)/ZnO nanorods/Ag nanoparticles hybrid coating on Ti implants for enhanced antibacterial activity and biocompatibility. Mater Sci Eng C Mater Biol Appl 2017 Oct;79:629-637.
- 53. Punjabi K, Mehta S, Chavan R, Chitalia V, Deogharkar D, Deshpande S. Efficiency of biosynthesized silver and zinc nanoparticles against multi-drug resistant pathogens. Front Microbiol 2018 Sep;9:2207.
- 54. Jafari A, Kharazi S, Mosavari N, Movahedzadeh F, Tebyaniyan M, Nodooshan SJ. Synthesis of mixed metal oxides nano-colloidal particles and investigation of the cytotoxicity effects on the human pulmonary cell lines: a prospective approach in anti- tuberculosis inhaled nanoparticles. Orient J Chem 2017;33(3):1529-1544 .
- 55. Beisl S, Monteiro S, Santos R, Figueiredo AS, Sánchez-Loredo MG, Lemos MA, et al. Synthesis and bactericide activity of nanofiltration composite membranes - Cellulose acetate/silver nanoparticles and cellulose acetate/silver ion exchanged zeolites. Water Res 2019 Feb;149:225-231.
- 56. Banu A, Rathod V. Biosynthesis of monodispersed silver nanoparticles and their activity against Mycobacterium tuberculosis. J Nanomedine Biotherapeutic Discov 2013;3(110):2.
- 59. Jaryal N, Kaur H. Plumbago auriculata leaf extract-mediated AgNPs and its activities as antioxidant, anti-TB and dye degrading agents. J Biomater Sci Polym Ed 2017 Nov;28(16):1847-1858.
- 60. Singh R, Nawale L, Arkile M, Wadhwani S, Shedbalkar U, Chopade S, et al. Phytogenic silver, gold, and bimetallic nanoparticles as novel antitubercular agents. Int J Nanomedicine 2016 May;11:1889-1897.
- 61. Singh R, Nawale LU, Arkile M, Shedbalkar UU, Wadhwani SA, Sarkar D, et al. Chemical and biological metal nanoparticles as antimycobacterial agents: A comparative study. Int J Antimicrob Agents 2015 Aug;46(2):183-188.
- 62. Choi SR, Britigan BE, Narayanasamy P. Ga (III) nanoparticles inhibit growth of both TB and HIV and release of IL-6 and IL-8 in Co-infected macrophages. Antimicrob Agents Chemother 2017;61(4).
- 63. Choi SR, Britigan BE, Switzer B, Hoke T, Moran D, Narayanasamy P. In vitro efficacy of free and nanoparticle formulations of gallium(III) meso-tetraphenylporphyrine against Mycobacterium avium and Mycobacterium abscessus and gallium biodistribution in mice. Mol Pharm 2018 Mar;15(3):1215-1225.
- 65. Olakanmi O, Schlesinger LS, Ahmed A, Britigan BE. The nature of extracellular iron influences iron acquisition by Mycobacterium tuberculosis residing within human macrophages. Infect Immun 2004 Apr;72(4):2022-2028.
- 66. Lu T, Wu Y, Zhao C, Su F, Liu J, Ma Z, et al. One-step fabrication and characterization of Fe3O4/HBPE-DDSA/INH nanoparticles with controlled drug release for treatment of tuberculosis. Mater Sci Eng C Mater Biol Appl 2018 Dec;93:838-845.
- 67. Saifullah B, Maitra A, Chrzastek A, Naeemullah B, Fakurazi S, Bhakta S, et al. Nano-formulation of ethambutol with multifunctional graphene oxide and magnetic nanoparticles retains its antitubercular activity with prospects of improving chemotherapeutic efficacy. Molecules 2017 Oct;22(10):1697.
- 68. El Zowalaty ME, Hussein Al Ali SH, Husseiny MI, Geilich BM, Webster TJ, Hussein MZ. The ability of streptomycin-loaded chitosan-coated magnetic nanocomposites to possess antimicrobial and antituberculosis activities. Int J Nanomedicine 2015 Apr;10:3269-3274.